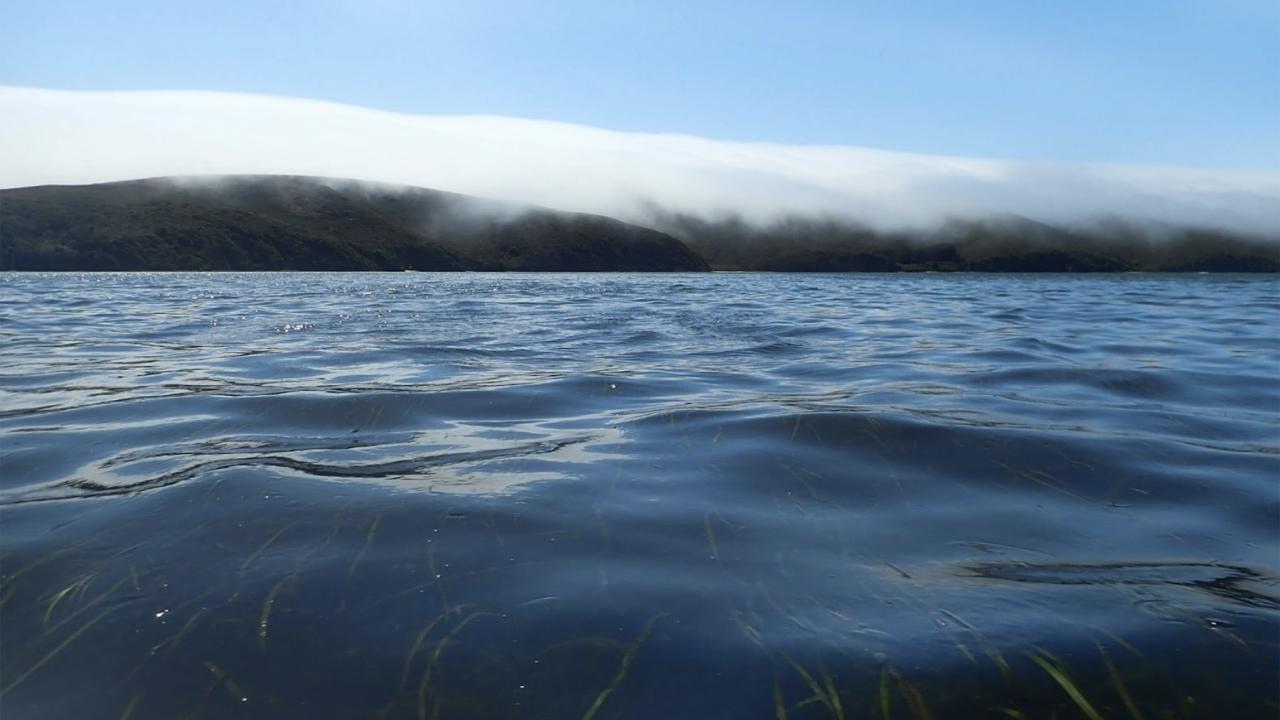
Ocean Acidification: Interdisciplinarity in Marine Science
Insights in the Bodega Ocean Acidification Research Group
Quick Summary
- Ocean acidification is a consequence of carbon emissions that has a wide impact on marine environments, including inducing food web shifts and threatening important shelled invertebrates.
- Ocean acidification is a highly interdisciplinary study that requires expertise across most major fields of science including biology, chemistry, physics, and geology.
- Seagrasses are important in combating ocean acidification and can help support sustainable sources of protein like aquacultured oysters.
When we think of the impacts of climate change, the words global warming, sea-level rise, and extreme weather will often come to mind. But another lesser-known, though equally serious, consequence of our anthropogenic footprint is the global acidification of our oceans. Fueled by the carbon dioxide we pump into the atmosphere, oceans worldwide have increased in acidity by 30% since the industrial revolution, damaging marine ecosystems and coastal communities, and the NOAA projects that the ocean acidity will nearly double by 2100 under current trends. Tackling the phenomenon, whose effects encompass biology, geology, chemistry, and physics, requires an interdisciplinary response. The Bodega Ocean Acidification Research (BOAR) Group unites researchers and scholars across different fields of science at UC Davis under a common goal of understanding ocean acidification–and has found solutions to its challenges.
A chemical context and why it’s significant
Ocean acidification (OA) occurs when carbon dioxide reacts with seawater. It combines with water molecules to form carbonic acid, which lowers pH by quickly dissolving into bicarbonate and hydronium ions (pH determines acidity by measuring hydronium; the more hydronium, the lower pH). Highly reactive, hydronium does whatever it can to bond with other ions, attacking and breaking down surrounding molecules to do so. This includes biological molecules that living organisms need to survive.
Life has evolved adaptations to cope with the pH of its environment, often even harnessing acidity to make essential molecules. But these adaptations are fine-tuned to specific pH ranges and easily break down when there’s too much hydronium, so a pH imbalance under OA thrusts marine organisms into an misaligned environment, often adversely affecting their growth and survival. There are a number of direct ramifications, but they collectively point to a unified consequence: a cascading effect on marine ecosystems, with impacts for industries like fisheries, medicine, and tourism.
Scientific research on OA is relatively new; the field only began to develop about 20 years ago when a 2003 study highlighted anthropogenic carbon’s role in the global phenomenon and coined its name. Though assisted by the exponential innovation of scientific technology, our understanding of OA remains incomplete. Another challenge in researching OA is its interdisciplinary nature. How a chemistry-based process affects everything else is driven by different processes across geology, physics, biogeochemistry, oceanography, ecology, physiology, molecular biology, and other disciplines.
The BOAR group was formed in 2008 by four UC Davis professors based at Bodega Marine Laboratory (BML)–Brian Gaylord, Tessa Hill, Eric Sanford, and now-Emeritus Ann Russell–when they realized they could unite complementary interests and expertise under one group. Reflecting OA’s interdisciplinarity, each professor comes from a different background of marine science: Dr. Gaylord is a biophysicist curious about how biotic and abiotic physics drives pH trends, whereas Dr. Hill is a geochemist interested in how marine communities geochemically react to acidification and affect climate change, while Dr. Sanford is a biologist exploring the eco-evolutionary relationships between coastal communities and acidifying oceans, and Dr. Russell is another geochemist with expertise in tracing acidification through stable isotopes. But in practice, roles are blurred when the professors are collaborating. “In really good teams like ours it is hard to define specific roles because we all play multiple roles and help each other out in different ways,” Hill explained. Joined by members of the professors’ independent labs and several postdoctoral scholars, the BOAR group is currently focusing on three particular consequences of OA:
Neurobiology and OA: predator-prey dynamics
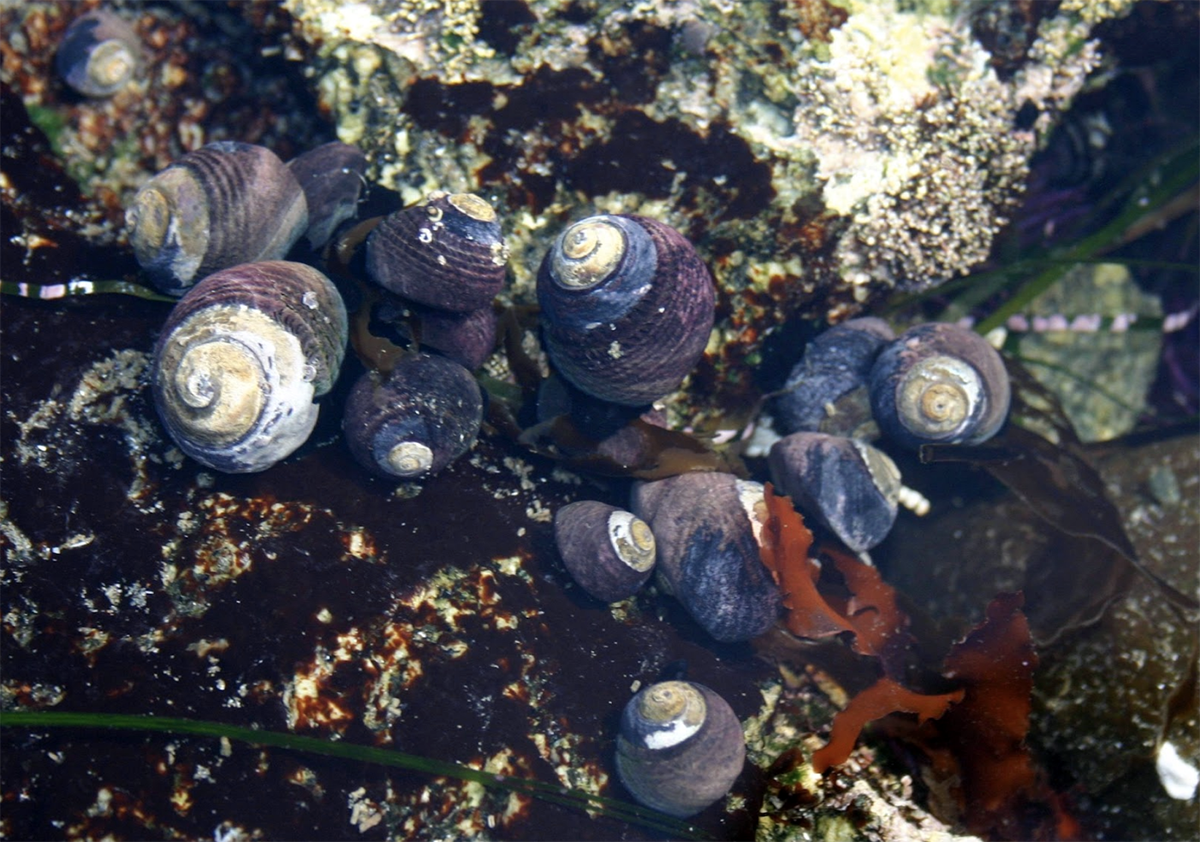
Decreased ocean pH can alter the senses in some prey species, which in turn changes their dynamics with predators that feed on them. Many marine organisms use environmental chemical cues to sense their surroundings, activating or deactivating neural pathways by picking up telltale molecules in the water. A prime example is the interaction between two animals common in California’s tide pools: the black turban snail (Tegula funebralis) and the ochre sea star (Pisaster ochraceus).
Black turban snails have chemoreceptors that detect chemical cues left behind by the voracious sea stars, activating a flight response that tells an algae-grazing snail to stop feeding and flee the tide pool. But in work led by then-graduate student Brittany Jellison, the BOAR group found that the snails don’t flee tide pools as usual when the waters are acidified. Drawing from earlier studies conducted on fish, the team hypothesized that change to the GABA pathway, a network of neuron receptors that eases fear when excited, is the culprit. Under a low pH-induced chemistry change, the receptors switch to a perpetual excitatory state, causing the snails to lose the ability to respond appropriately to sea star cues. Consequently, this allows the sea star to better catch the snails, as documented in several studies including one published in 2022.
The snails aren’t disappearing. Gaylord explains that because of the inhibited flight response, snails that avoid consumption are able to eat more algae for longer periods of time. This counteracts the impact of increased predation. But black turban snails are not usually the preferred prey of ochre sea stars, and this might change as snails become easier to catch, affecting how the sea stars prey on other animals and changing their ecological roles in a cascade effect. “What we are seeing [are] essentially food web shifts in these systems under ocean acidification,” he added. How exactly these food webs are going to change is an emerging topic that requires further research on community-wide reactions to individual OA-altered predator-prey relationships.
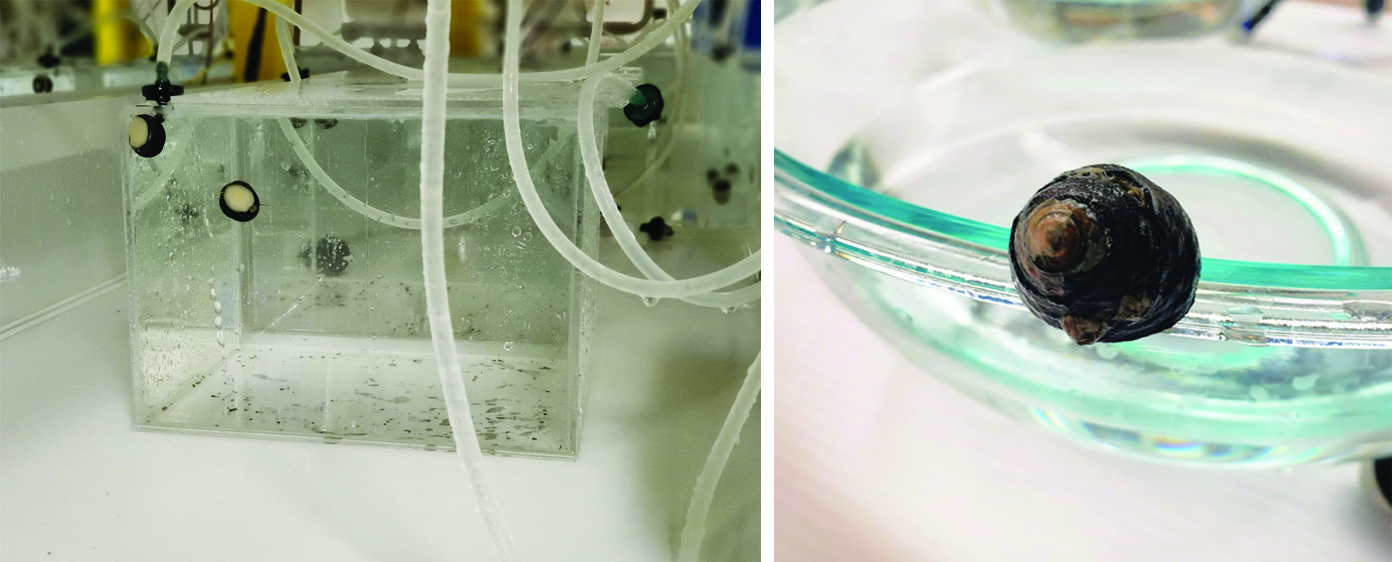
Two of Gaylord’s graduate students, Alisha Saley and Ashley Smart, are currently expanding the research on black turban snails at BML. Saley is investigating similarities between the OA snail-sea star interaction and other predator-prey relationships, while Smart is working on teasing out the neurobiological mechanisms of these relationships. The two experiment with snails by conditioning them to different levels of pH, artificially replicated to match natural OA situations through sequential injections of carbon dioxide. Saley simulates each snail’s encounter with a different predator species while Smart experiments with chemicals targeting suspected neurobiological mechanisms. Research is ongoing, but preliminary results already shed some light. Smart’s data suggests that the GABA pathway is potentially more complex under OA than previously hypothesized, while Saley is finding evidence of similar impacts of OA on flight responses to different snail predators that depends on how strong the snail’s response to a given predator is under normal pH conditions, potentially allowing ecologists to extrapolate to other predator-prey interactions up the food chain.
Abalone pH resilience for sustainable aquaculture
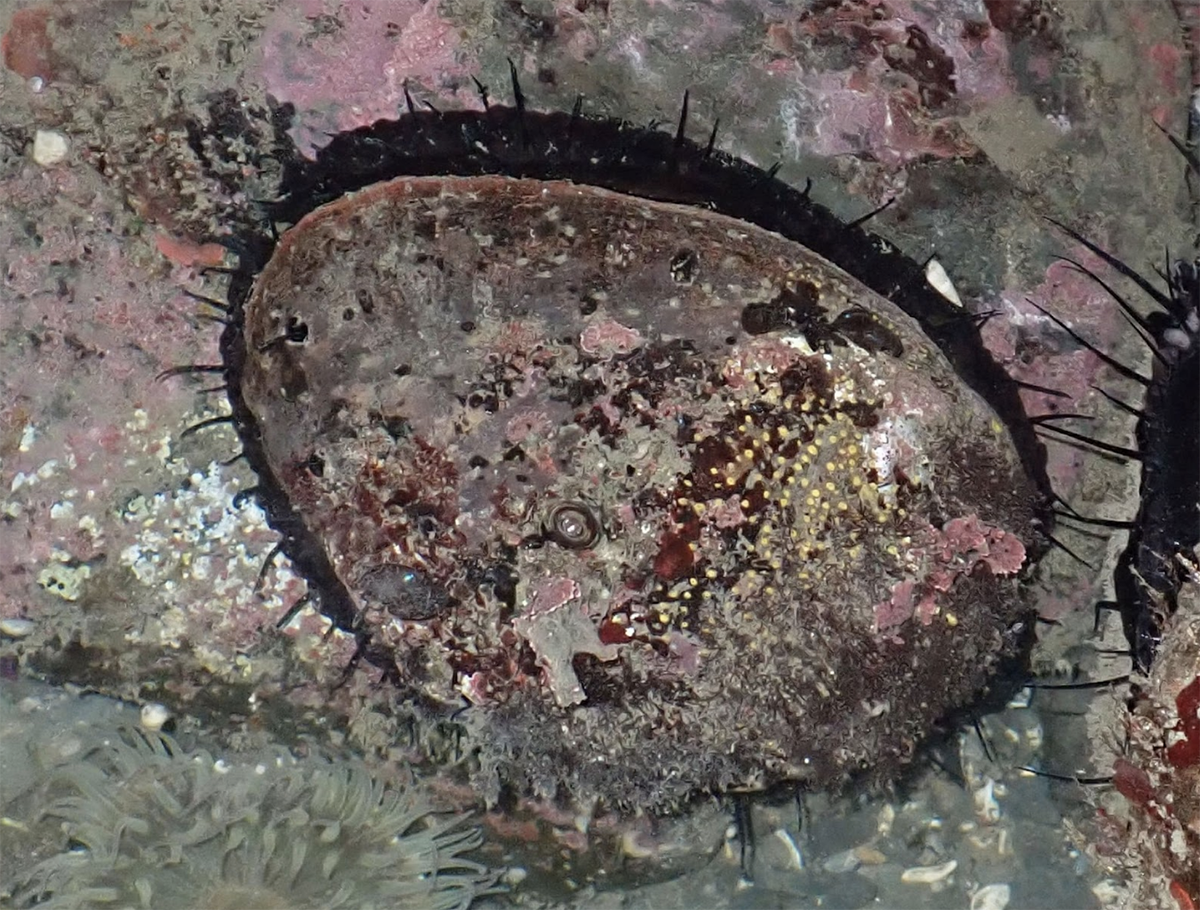
California’s abalone are highly valued by both humans and nature. It is an ecologically important species in coastal ecosystems and a prized delicacy around the world. Abalone aquaculture, which developed as overfishing decimated native populations, has proven itself to be a major source of sustainable protein–farmed abalone live entirely off abundant and fast-recovering local algae and discharge minimal harmful waste.
Abalone use calcium carbonate to build their shells. But in an acidified setting, hydronium steals carbonate. They snatch the available carbonate underwater, giving abalone less resources for shell-building, and dissolve existing shells to extract more carbonate. The abalone is forced to invest more energy in coping with these losses, which becomes a life-and-death scenario during infancy. Abalone are born as free-swimming larvae unable to feed themselves. Instead, they rely on a prepackaged lunch box of lipids provided by the mother for the first weeks of life. Under the stresses of OA, young abalone use up their store more rapidly. The lipids run out too early and the abalone starves to death. As a result, abalone farms are threatened with increasing mortality as oceans further acidify.
But the BOAR group noticed that populations of red abalone (Haliotis rufescens), a popular cultivated species, are doing just as well in Northern California as populations in Southern California. This is significant because NorCal is a site of significant upwelling, where winds and currents bring extremely acidic deep-sea water to the surface. The team hypothesized that upwelling-experienced NorCal red abalone are more resilient to low pH than their SoCal counterparts.
They tested these ideas in an experiment led by postdoctoral scholar Dan Swezey, which simulated acidified aquaculture settings in three generations of wild red abalone from Mendocino County, a site of strong upwelling, and captive abalone from a Santa Barbara farm. The results published in 2020 confirmed their suspicions: the Mendocino abalone did not seem to be affected by the acidification, while premature deaths by the Santa Barbara abalone rose by about 50% under acidified conditions. Upon closer inspection, the BOAR group discovered that Mendocino abalone larvae were born with a larger lipid lunch box and a slower growth rate to conserve their fuel. “It seems like the mothers have evolved to invest more energy in [their offspring],” Sanford explained, pointing to genetic adaptations that make this possible. Introducing OA-resilient strains into abalone farms can support aquaculturists with increased sustainable yields, though with a trade-off. The decreased growth rates mean longer wait times before abalone hit the market, an economic challenge for farmers competing with other highly productive but unsustainable industries.
Reversing ocean acidification with seagrasses?
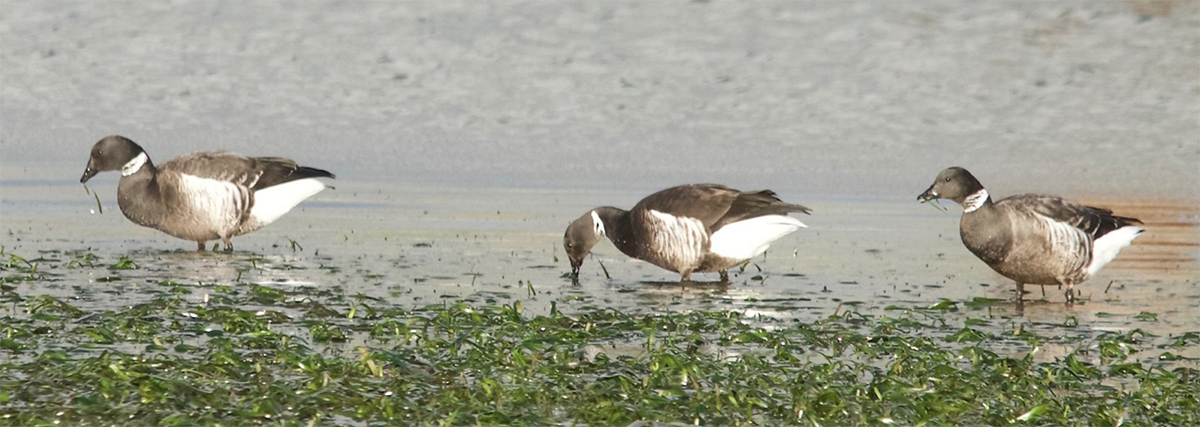
Seagrasses grow in dense meadows in estuaries along the California coastline. At first glance they may appear as little more than vast fields of unremarkable slimy green stalks, but these plants secretly hold a profound role in shaping our oceans. They are ecosystem builders that provide food and refuge for marine life with diversity that rivals coral reefs and they protect coastlines from storms by absorbing wave energy. Seagrasses have buried within their leaves another secret that has caught the BOAR group’s total attention: the ability to offset ocean acidification.
How can a bunch of grass help combat centuries of underwater climate change? Part of the answer can be found in their green coloring. Seagrasses are heavy photosynthesizers that absorb large quantities of carbon dioxide from the water, forming carbon sinks that sequester the carbon, or prevent them from dissolving into acidic components. This in turn temporarily deacidifies the surrounding water. Other marine photosynthesizers such as giant kelp share super carbon-sequestration capabilities, but seagrasses are exceptional in their ability to ameliorate acidic seawater.
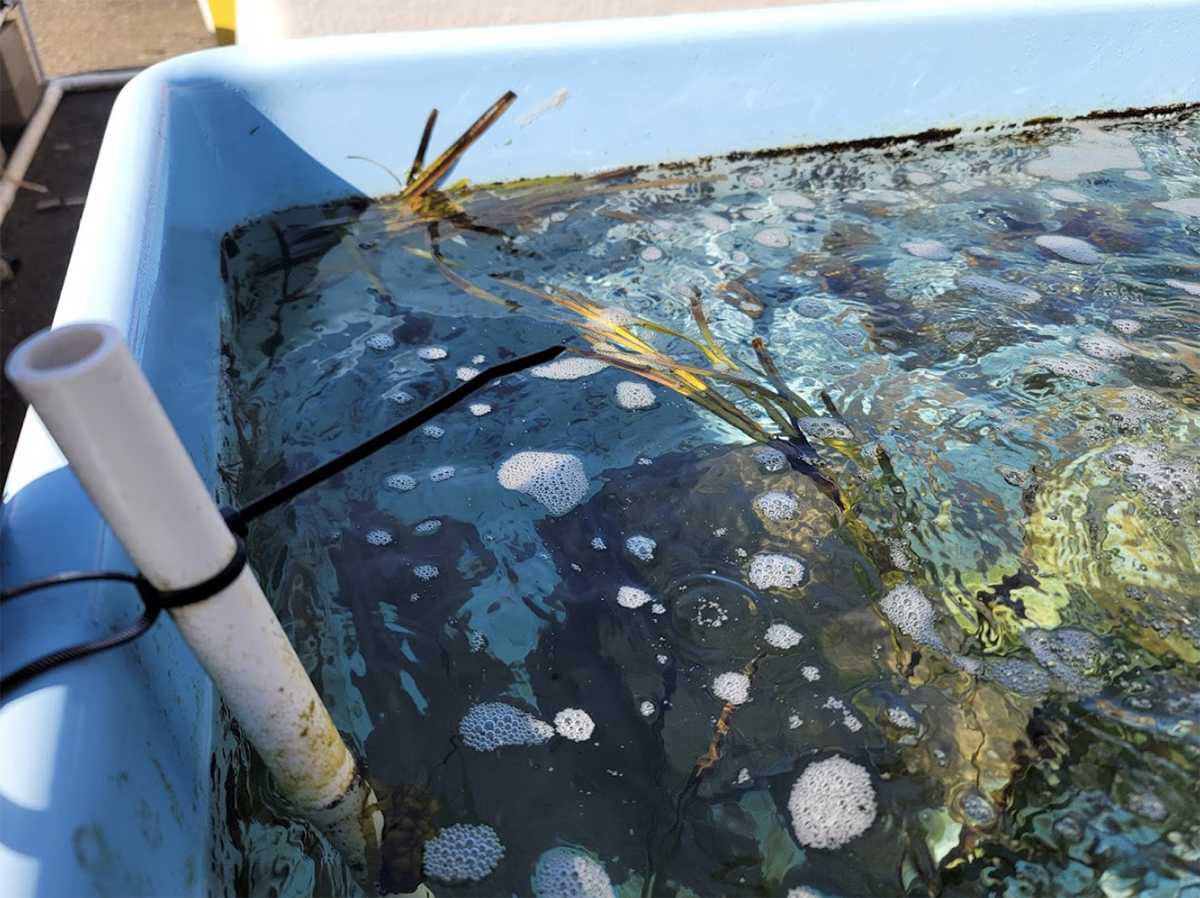
To find what makes seagrasses so good at this, the BOAR group led by postdoctoral scholar Aurora Ricart and then-graduate student Melissa Ward conducted a study published last year that examined pH trends of seven meadows of the common eelgrass (Zostera marina) over a six-year span. This was not an easy task: measuring pH required specialized sensory equipment resilient to the elements, something that was not readily available at the time. The team collaborated with the Monterey Bay Aquarium Research Institute to use a recently developed pH sensor that could measure with high precision and weather out storms while remaining in eelgrass beds for weeks to months at a time. Several technical difficulties in the form of sensor failures and glitches were experienced, but in the end the group overcame this challenge and collected all of the needed data. The results were astounding: the eelgrasses raised the surrounding pH 65% of the time (usually during spring growing seasons) by up to 30%, essentially reverting to pre-industrial levels in what Gaylord described as “turning the clock back on the seawater acidification process.” Moreover, these reversals were conserved at night when photosynthesis is not active, ensuring a stable maintenance of deacidified waters.
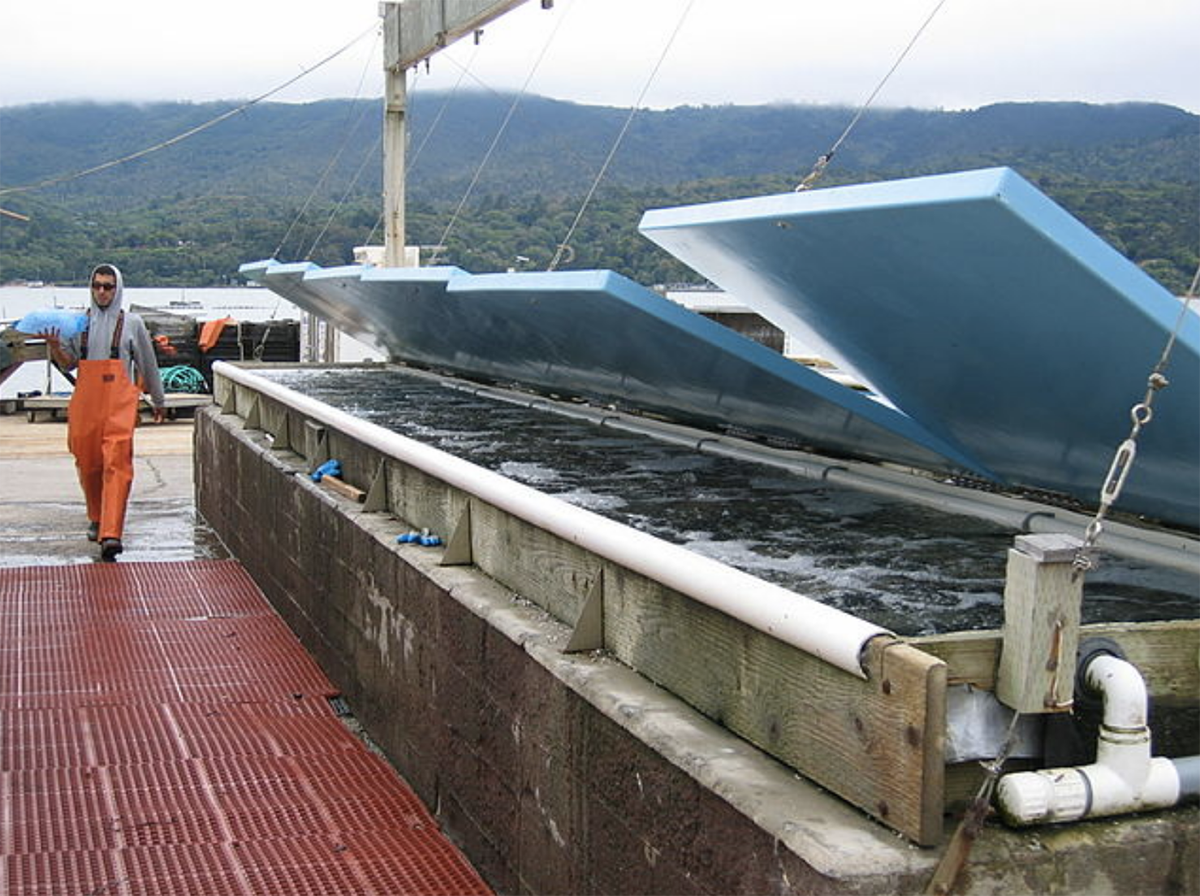
This discovery can provide substantial benefits locally, especially in aquaculture. One such application is in the commercial oyster industry, which operates open-water farms along the West Coast and shares similarities to abalone in sustainability. Like abalone, oysters face OA challenges related to their calcium carbonate shells. But if seagrass beds are present near these facilities, the team hypothesized that they, as Sanford puts it, “could help the oyster growers by having more favorable conditions for the oysters to produce their shells.” To test this, Ricart led the BOAR group in another study. They obtained juvenile oysters from Hog Island Oyster Company, which has oyster farms in Humboldt County and Tomales Bay (situated between San Francisco and Bodega). Taking them back to BML, Ricart tested whether raising the oysters with eelgrass affected their growth under OA. The results were another success as they found that very high eelgrass biomass increased oyster shell growth rates by up to 40%. Supporting oysters through seagrasses goes beyond green seafood. The oysters themselves provide vital services to surrounding ecosystems as underwater custodians. They filter water by extracting and sequestering dissolved substances like carbon and nitrogen, which helps mitigate harmful algal blooms that create oxygen-devoid dead zones. It is thus in our best interest, both ecologically and economically, to restore and preserve existing threatened seagrasses to facilitate our best tools against OA.
What it takes to understand ocean acidification
As a field that commands interdisciplinarity, studying OA requires skills and knowledge across the sciences to an extent that many scientists don’t typically reach. In fact, each of the BOAR members themselves had to learn new foundations outside their comfort zone. Sanford recalled his own experience:
“I’m not someone with a strong background in chemistry in particular and so learning about carbonate chemistry was sort of a whole new dimension for me. As someone who's more of an ecologist and a biologist, those aren't things that I really thought about in my research much before teaming up with Tessa and Brian so I think working on ocean acidification has challenged all of us to think about disciplines that we don't normally work in.”
To aspiring undergraduate researchers, Sanford emphasized building a strong foundation in both marine science and other fields such as ecology and evolution before focusing on ocean acidification: “make sure you have a solid foundation in all of these disciplines so that when you turn your attention to understanding your specialization you’re not just looking at it through a very narrow lens but you're thinking about all the different processes that might involve or might influence how organisms respond to ocean acidification.” To build that foundation, he recommends a two-prong approach. First, undergraduates should take all the necessary foundational courses in biology, chemistry, mathematics, physics, and other disciplines. For Marine and Coastal Sciences students, all are accounted for in their major requirements. Second, students should develop research experience by involving themselves with one of the many lab opportunities UC Davis has to offer. To the latter, Gaylord stresses that basic research skills is the emphasis of lab involvement, and so students need not worry about getting into one particular lab. Hill also emphasized the importance of following your curiosity, staying up-to-knowledge by reading scientific literature when available, and reaching out to potential mentors.
About the Author:
David is a first-year student studying marine and coastal sciences. Driven by a lifelong fascination with ecosystems and natural history, he hopes to dedicate his undergraduate years to research and promoting awareness about current oceanic issues alongside his studies. He also likes to draw and build Minecraft worlds with friends.